Bio-Hybrid Systems for Ecosystem Level Effects
This article is an edited version of the original publication:
Asya Ilgün, Kostadin Angelov, Martin Stefanec, Sarah Schönwetter-Fuchs, Valerin Stokanic, Jutta Vollmann, Daniel N. Hofstadler, Martin H. Kärcher, Heinrich Mellmann, Volha Taliaronak, Armands Kviesis, Vitalijs Komasilovs, Matthias A. Becher, Martina Szopek, David M. Dormagen, Rafael Barmak, Erol Bairaktarov, Matthieu Broisin, Ronald Thenius, Rob Mills, Stamatios C. Nicolis, Alexandre Campo, Aleksejs Zacepins, Sergey Petrov, Jean-Louis Deneubourg, Francesco Mondada, Tim Landgraf, Verena V. Hafner, Thomas Schmickl; July 18–22, 2021. “Bio-Hybrid Systems for Ecosystem Level Effects.” Proceedings of the ALIFE 2021: The 2021 Conference on Artificial Life. ALIFE 2021: The 2021 Conference on Artificial Life. Online. (pp. 41). ASME.
Read the original paper in open access:
https://doi.org/10.1162/
Introduction
Life exists at the edge between order and chaos (Langton, 1990; Lewin, 1999). It needs boundaries to conserve its vital structures and flexibility to grow and adapt (Levin, 1998). The cell, as the basic unit of all life, exemplifies this idea: it is surrounded by a membrane which not only separates its self-maintaining machinery from a potentially hazardous environment but also allows for a controlled exchange of substances like water and nutrients (Cavalier-Smith, 2006, Lombard, 2014; Ribatti, 2018). While boundaries are essential to maintain the structures of a living system, overcoming them may also lead to a major step in the evolution of this system.
The transition from unicellular to multicellular organisms, the evolution of sexuality, symbiotic relationships between species, maybe even the emergence of the human mind – all these are examples of how breaking through previously existing limitations can open a whole new dimension of possibilities (Gould, 1994; Maynard Smith and Szathmary, 1995). Living organisms are the most complex systems we know of. However, in the past decades, man-made technologies have become more and more sophisticated with ever-increasing speed. Recent significant advances in computer science, artificial intelligence, microtechnology and robotics now allow us to design devices that are capable of interacting with living systems in a more meaningful way than ever before. The interactions between artificial devices and living organisms could become important and powerful bio-hybrid agents for ecosystem stabilization and support, as is for example the main objective of the technology developed in HIVEOPOLIS (EC, 2019), see Figure 1 for an overview of its system layers and components.
Such bio-hybrid systems are composite dynamical structures that merge and blend biological organisms with technological devices into a new functional unit: a novel bio-hybrid agent. Guiding a living organism based on a stream of information enriched by human knowledge (threats, forecasts, coordination efforts) can help this organism to thrive and enhance its chances to survive and reproduce. With social insects, such as honeybees, this step can even be elevated onto enriching a whole colony, which is often seen as a “superorganism”. It may well turn out that such bio-hybrids are going to be the next evolutionary step in the history of life on Earth by overcoming the border between the animate and the inanimate world. However, in order to actually be used outside of the scientific laboratories, to have the chance to be released into nature and to evolve, bio-hybrids not only have to demonstrate their performance within the system they are directly applied to, but also have to be accepted by human societies and must prove their functionality within the context of the whole ecosystem they are exposed to. In this paper, we provide a general outline how bio-hybrid systems may be developed, communicated and applied. We underpin our arguments with examples from a currently running EU-funded project on the development of a technologically augmented future honeybee hive.
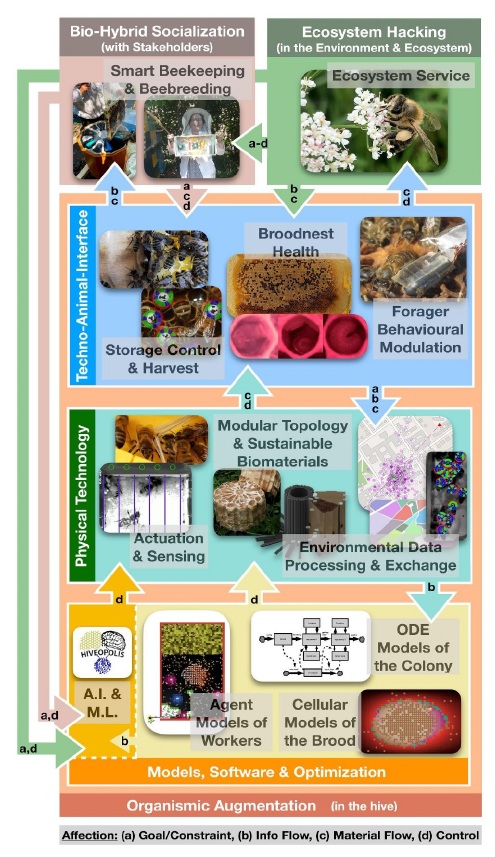
Related Work
Various approaches to develop animal-robot-interaction have been explored (see Romano et al., 2019). These include locomoting robots (e.g., cockroach robots, Halloy et al. 2007; fish robots, Faria et al. 2010, Bonnet 2017, Worm et al. 2017; or artificial sheepdogs, Vaughan et al., 2000). Devices mounted on individual cows (Butler et al., 2006) could induce herd-level responses.
Developments relating to honeybees concentrate more on measurement (reviewed in Meikle & Holst, 2015) but notable exceptions – all targeting laboratory use so far – are the dancing robots that interact with forager honeybees (Michelsen et al., 1992, Landgraf 2013), and static arrays of actuators that locally sense and emit heat and vibrations to modulate honeybee groups (Griparic et al., 2015, Stefanec et al., 2017, Mariano et al., 2018).
Ecosystem Level Bio-Hybrid Systems
Our scientific approach to design bio-hybrid systems that can tackle the aforementioned challenges requires a set of transdisciplinary research tracks in several domains of science. The required research is given by the requirements of the system displayed in Figure 1. Three major steps have to be undertaken in order to establish bio-hybrid systems: first, the technology has to be developed and tested (“Organismic Augmentation”), it then has to be accepted and seen as beneficial by human society (“Bio-hybrid Socialisation”) and finally it has to prove its validity when deployed at an ecosystem level scale (“Ecosystem Hacking”). To illustrate our argumentation, we provide an example for each of these three concepts from the research project HIVEOPOLIS, which already allowed us to develop several implementations to test the feasibility of our conceptual framework.
HIVEOPOLIS aims to engage an expert community in the emerging field of bio-hybrid systems to research a path towards a novel living technology: It will employ a new paradigm that embeds technology (sensors, actuators, robots, algorithms) into a living superorganism (honeybee colony) to create a new symbiotic life form with novel characteristics concerning stability, resilience, robustness, efficiency, applicability, controllability and scalability. HIVEOPOLIS technology employs honeybees as a symbiont to technology, as they are important pollinators of wild flowers and crops and hence play a central role in ecosystems and in human food production. For example, by integrating technology into the bees’ dance-based recruitment system (von Frisch, 1965) while simultaneously strengthening brood production to boost future forager workforce and foraging demand, we aim at increasing ecosystem services via three lines of biohybrid technologies:
1. Organismic Augmentation
Honeybees are not well adapted to the stressors of our anthropogenically altered world (Youngsteadt et al., 2015; Belsky & Joshi, 2019). However, a set of modern technologies can upgrade their colonies by augmenting them with new abilities. To achieve this, the first principle in our HIVEOPOLIS paradigm is “Organismic Augmentation” (OA), a novel paradigm for embedding technology into societies of living organisms. Establishing information flow and feedback loops between these components can enhance the performance of the biological and artificial agents, constituting a novel biohybrid superorganism.
We develop a technologically augmented futuristic beehive that supports the well-being and survival of bees in harsh, industrialized and urbanized conditions. This includes technology to modulate foraging targets (Sec 3) and active closed-loop defense against honeybee parasites that could potentially inflict economic harm counted in billions of Euros (Potts et al., 2016). The research also includes incorporating predictive mathematical models and the injection of data unavailable to bees naturally (e.g., weather forecast, satellite data, etc.).
Organismic augmentation consists of components from three system layers: models & software, physical technology and the specific techno-animal-interface. These components are connected through various interactions: the software controls certain parts of the physical technology, the hardware components collect data and change physical parameters at the techno-animal interface, which dictates the requirements for the design of the physical hardware. The animals themselves not only provide information, but also modify the physical hardware (for example, by depositing wax, nectar and pollen).
In order for OA to be effective, some basic principles must be considered at the interface between technology and nature: The materials used, the physical embedding of the technical enhancements and the measurement of physical processes in the hive must not trigger negative effects on the physiology and natural behaviour of the animals. If a particular animal behavioural response is to be elicited in the system, we must ensure that all consequences of the behavioural response cascade to the stimulus are known. Another important principle to ensure a gentle technology integration is to not massively exceed the magnitude of physical stimuli as they occur in nature. Behavioural responses of animals should occur as natural responses to artificially produced stimuli, as if these were natural cues of their conspecifics.
Augmented Brood Nest.
The paradigm of OA can be explained via the example of an augmented brood nest, which is one component within the HIVEOPOLIS bio-hybrid superorganism. In the brood nest, the queen lays eggs into individual cells, which will develop into larvae and later pupae before they hatch as adult bees. In this area, temperature regulation plays a crucial role (Stabentheiner et al., 2010) because bees only develop healthily within a specific temperature range.
The brood nest system (Fig. 2) will autonomously monitor the health status and parasite infestation levels of the brood, determine colony growth, regulate the brood nest temperature and influence the behaviour of the colony via temperature stimuli. Informed by accurate weather forecasts, our system is designed to reduce brood cannibalism and to strengthen the brood nest at the most beneficial periods of time, while in parallel, we aim to modulate the queen’s egg laying rate via subtle artificially induced temperature stimuli. Introducing these feedback loops between the animal society and external information, via models and technology, is a key exemplar of the organismic augmentation paradigm.
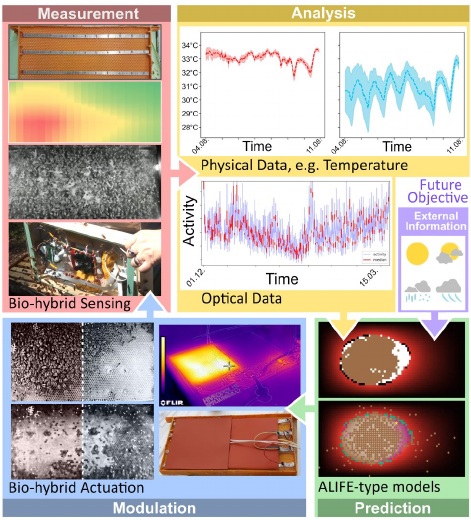
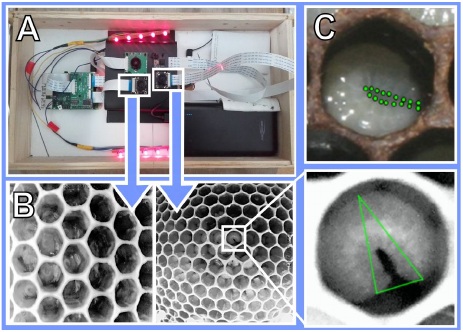
Non-invasive Survey of Brood Development.
For a healthy bee colony an optimal development of the brood is essential. To be able to observe potential negative environmental influences on brood development, our non-invasive method for contactless determination of age and development of honeybee larvae allows a real time close-up look into the brood nest area. This allows a timely counteraction against various stressors that can cause significant colony losses.
Our method aims to determine morphometric features by detecting the inner boundary of the larvae and by consecutively measuring the triangle that is formed by the two ends and the adjacent midpoint of the larvae’s outer circumference (Fig. 3C). To minimize disturbances of the colony during taking pictures we build a prototype camera module (Fig. 3A) that is custom built into the physical structure of the hive. This is made of commercially-available components and is energyautonomous. We installed different cameras to get varying sectors and details of the comb (Fig. 3B). This allows to monitor whether or not the size development is appropriate for the given age, in order to detect stressor-induced growth deficits. The data from these detailed automatic observations flows into predictive models that inform other modules how to respond and are thus a key part of the novel feedback loops within the bio-hybrid superorganism.
2. Bio-Hybrid Socialisation
On the one hand, the best technological augmentation of beehives will be ineffective if it is not applied by the significant stakeholders. On the other hand, many data sources and information flows that are available to those stakeholders are mostly inaccessible to natural bee colonies today. To address these two premises, the second principle in our approach is the “Bio-Hybrid Socialization” (BHS). This novel set of methodology and technology is developed to allow natural organisms (via their technological augmentations) as well as whole ecosystems (via their embedded bio-hybrids) to participate in an information exchange with our human society of stakeholders. Organisms, whole ecosystems, and humans can engage and influence each other thus becoming a radically new biohybrid “social cyborg” in the form of a human-technoorganismic biocoenosis. The critical contribution of BHS is for human stakeholders to adopt bio-hybrid methodologies.
Exploring New Hive Topologies.
Ecologists have long recognized that the physical structure of a habitat influences population biology (Bell 1991). Our key design question is the measure of the physical structure’s combined adaptability to the needs of honeybees, humans, and to the environmental conditions. There are many competing design goals regarding the performance of the hive structure, while our primary criterion is the measure of colony health and wellbeing.
Our individual hive modules are arranged around a central core in a radial symmetry instead of the parallel arrangement found in common beehives. This mainly eases human intervention to individual comb modules, without having to open the whole hive. It additionally provides a central computation core with hardware and software with high connectivity to the surrounding comb modules. To ultimately assess the suitability and quality of a specific design, it requires a thorough evaluation of the direct and indirect impacts of:
- function: the collection of activities and uses that the hive and its spaces serve
- form: geometry of the hive and the spaces within it.
- organisation: the spatial relationships among inner spaces (and bee circulation paths)
Hive-Topology Evaluations and Benchmarks.
Three “startopology” hive prototypes (Fig. 4A&B) were populated on 02.07.2020, each with 2 kg of bees and four brood frames. Three “mitochondria topology” hive prototypes (Fig. 4C&D) were populated on 23.07. The aim of both experiments was to observe the queen mobility and brood distribution inside the novel hive designs. The experiment reported here aims to investigate which of the two alternative topological designs will be better accepted by the bees.
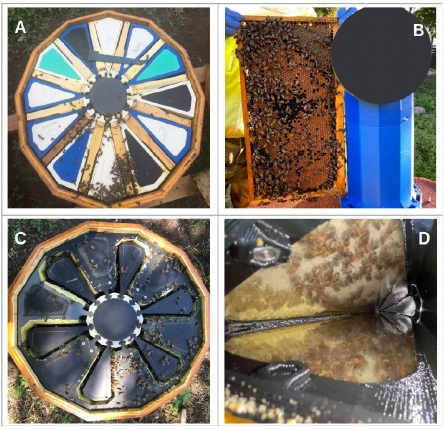
The star topology design permits standard frames to be removed from the hive and to photographically measure how the bees utilize the hive space, and the resulting ratios of brood:honey. In contrast, the mitochondria topology is based on the concept of a continuous wax foundation, thus impairing pulling out comb segments to be examined and measured. Instead, we therefore installed modules with transparent walls to observe the areas of the hive where the bees were active, either tending to brood or the colony’s food supply. This way, we visually determined the amount of space utilized by the bees and assessed the colony development. Each hive was supplied with 1 liter of sugar syrup solution (1:1 water to refined sugar) every day for the first three days followed by regular 7-day feedings. To assess the colony status we performed thorough visual assessments (Fukuda, 1971) of how much comb area of the hives was utilized in each design every 12 days (Fig. 4B&D). Figure 5 shows the colony development in both topologies: All 6 colonies developed well and sustained or even grew over time, with the “star” topology hives performing better in terms of colony development. More detailed observations will be possible once our camera modules (Fig. 3) are integrated into these hives.
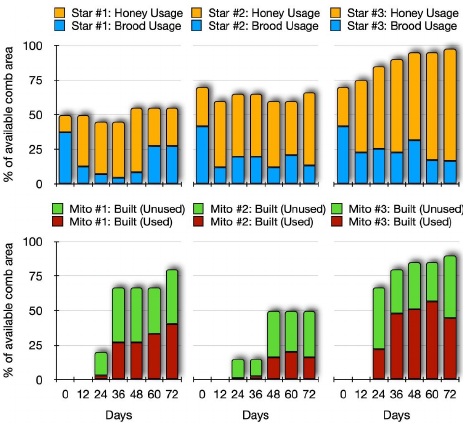
Fractional honey harvesting system.
Fractional honey harvesting system. A novel approach towards a “fractional honey harvesting system” is envisaged in our HIVEOPOLIS paradigm. The functionalities implemented in the storage component are to monitor, analyze and control nectar flow inside the beehive. Our honey harvesting system will allow a selective fractional (less than one frame) extraction of honey from the hives without the disturbances induced by a classical physical beekeeping intervention. The goal is not only to facilitate one of the most labour-intensive parts of beekeeping, but also to establish functional control of the colony by actively controlling the availability of honey and nectar. Such a proactive control of resource availability will allow us to influence colony decision making in a sustainable way. Early extraction system prototypes are currently in development and will be tested in the forthcoming active bee seasons.
Community Hive.
We established a special hive topology (Fig. 6) to bring the features of a flat “observation hive” into a 3D form factor (cube), thus combining the energetic advantages of this shape and having multiple observation points (e.g., for sensors, cameras) at the outer surface and in the central core of the hive. This hive is designed to serve several purposes: i) To present HIVEOPOLIS technology to stakeholders in order to connect science to the public; ii) To benchmark HIVEOPOLIS technology prototypes; iii) To exemplify the future hive with reduced complexity and costs.
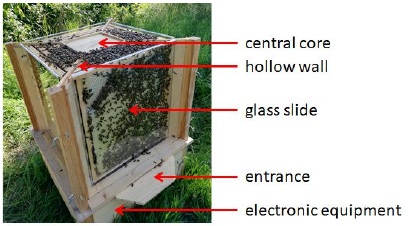
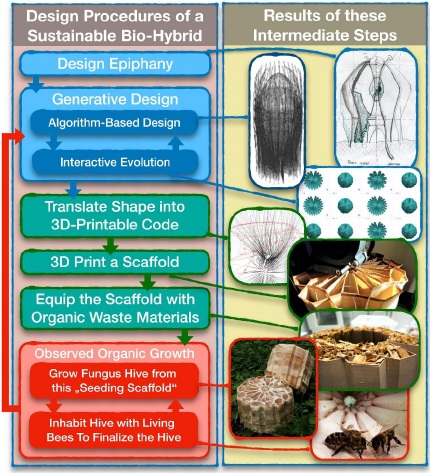
Design-to-Biofabrication Method for a Sustainable
Biohybrid.
One important design aspect in our concept is the biological augmentation processes used both in construction and operational phases of the HIVEOPOLIS components. We grow naturally-existing complexes (such as fungal networks, or mycelia, that are composed of chitin, cellulose, protein, bioactive substances) in and around 3D-printed scaffolds containing lignocellulosic waste substrates. Previous work has used desiccated mycelia as a sustainable replacement for styrofoam beehive enclosures (Turner 2017). In contrast to that, we use living mycelia to create a responsive, self-repairing structure with life-like behaviour, emerging from geometries (form) instead of from matter (substance). This branch of our research paradigm combines a material–fabrication-based design algorithm – that Oxman (2007) also calls “digital crafting” – and the implementation of a thorough, empirical understanding of fungal growth as material building processes. The fungal materials and biofabrication community is growing, comprising industry, biotech start-ups, academic institutions, and most importantly open source communities (Elsacker et al., 2019) in which we position HIVEOPOLIS biohybrid societies.
The deposition-based 3D printing (FDM) technology permits the production of highly porous yet robust artefacts to be constructed from organically driven materials (Ilgun and Ayres, 2016). Our digital design technique yields an increased porosity thus an increased surface area for mycelium coverage which is an important measure for the strength of hive material (Yang et al., 2017). Here we describe a hybrid construction method for sustainable biohybrids (Fig. 7).
1. Generative Digital Design: We constructed the conceptual design sketch with an algorithm-based parametric model that generates the hive geometry and translated it into a single point cloud forming a continuous polyline, using a visual programming language (Grasshopper3D). This polyline defines the 3D-printer’s toolpath, thus where the material would be deposited. This method provides time and energy efficiency both for computation and production, as well as opening up new aesthetic possibilities. Within the same design model, a Cluster-Oriented Genetic Algorithm (Parmee, 1996) rapidly explores a diverse design space. This algorithm tackles quantitative criteria that compete with each other, such as the inner volume of the whole structure for bee inhabitation as well as the wall chambers to be filled with mycelium substrate, and the length of the polyline as a measure for needed raw material. Biomorpher, an Interactive Evolution plug-in for Grasshopper3D, allows us to interact with the algorithm during the evolutionary process via its GUI providing cluster representatives in 3D. Thus we can select parents with qualitative criteria in each generation (Harding et al. 2018). The selected scaffold geometry is translated into a 3D printable code (g-code).
2. Materialization: The scaffold is printed with a large-scale FDM 3D-printer. We used a composite filament (Growlay™) made of cellulose particles, a water-soluble synthetic polymer (PVA) and a backbone polymer. PVA is soaked out from the printed components. This increased the needed porosity and minimized the amount of synthetic chemicals within material, although weakened the layer adhesion and thus structural scaffolding capacity of components.
3. Integration of living: In a sterile environment, we fill the vertical cells of the 3D printed components’ walls with lignocellulosic waste substrate which is pre-inoculated with spores of the selected mycelium strain. Our most recent prototype is inoculated with Trametes versicolor mycelia for its well documented medicinal properties and pollutant degradation capabilities (Stamets, 2005; Jong and Field, 1997; Hobbs, 2005). Next, hive components are stored in plastic boxes with almost no air exchange, in a dark and warm spot, until the mycelium network is visibly dense and well distributed in and on the scaffold. It takes approximately 2 weeks until we take the components out to the field and assemble them on-site for further outdoor growth and adaptation. During this phase, mycelium grows towards the ground, creating a foundation and naturally welding the stacked hive components to each other, leaving the top one operable for bee colony introduction.
3. Ecosystem Hacking
Based on the technologies and methodologies described above, HIVEOPOLIS aims to go beyond augmenting the bees in the heart of their colony. In addition, it also aims to support the hive’s interaction with its surrounding ecosystem, thus to employ technology to influence the bees that physically interact with the outside environment. In order to do so, the third and final ingredient in our paradigm is “Ecosystem hacking” (EH): an augmentation of whole biological communities by introducing novel players and by establishing novel ecological relationships, in order to change the performance and/or the stability of the whole ecosystem in a favourable way. In HIVEOPOLIS we employ EH to achieve different goals. The following list summarizes potential use cases of Ecosystem Hacking using HIVEOPOLIS technology in the future. The different ideas are detailed in the remainder of this section.
- Modulate honeybees’ colony growth and their activity to
increase their winter survival. - Autonomously detect and treat against ectoparasites.
- Detect detrimental foraging sites affected by herbicides, fungicides, insecticides, and other toxins by turning colonies into environmental sensors.
- Prevent bees from foraging at toxic/dangerous sites and guide them towards safe foraging places.
- Create a natural food reserve for wild pollinators by preventing the local bees from foraging there.
- Improve the pollination service by guiding foraging.
- Strengthen urban ecosystems service by making urban beekeeping more accessible for a modern lifestyle.
- Let beekeepers know where their honey is coming from.
- Share data (e.g., info on forage sites) between hives.
Augmenting the Foraging Activity.
Honeybees exhibit a rich repertoire of communication behaviours among which the ‘waggle-dance’ stands out in the animal kingdom as a unique one-to-many symbolic language: After visiting a rewarding forage site, the foraging bee can perform a stereotypical motion inside the hive that encodes both the direction and distance to the advertised site. Interested bees can decode this information by following the dance and can find the location in the field (von Frisch, 1965). Experiments in observation hives have shown that it is possible to automatically detect and decode these dances (Wario et al., 2017), see Fig. 9. While previous solutions required a large observation setup with the dance floor exposed to an external camera and lighting system, we will build a smaller system that can be integrated into a closed hive to automatically monitor the dance and forage activity, making a HIVEOPOLIS system an environmental sensor that constantly collects information about the availability and quality of forage sources around it. Combined with external mapping data about the surroundings, this can give beekeepers an early-warning system on adverse conditions affecting the energy intake of their colonies.
However, to fully integrate several HIVEOPOLIS hives into a bio-hybrid super organism, we also have to guide the foraging activity of the colonies. Previous work has shown that biomimetic robots can imitate the waggle-dance and elicit following behaviours by bees (Landgraf et al., 2018), see Fig. 9. Integration of such a robotic agent will allow the HIVEOPOLIS hive to establish bi-directional communication with the bees: The hive will be able to guide foraging activity to favourable food sources and can forward information from the beekeeper or environmental maps directly to the bees. The integration of dance decoding and a dance robot also allows honeybees to communicate foraging sites not only to their nestmates but also to different colonies by relaying observed dances from other colonies with the dance robot.
To fully enable the HIVEOPOLIS hive to steer the foraging activity of the colony, it also needs the means to suppress dances pointing to unfavourable locations. Artificial vibrations have been shown to modulate honeybee movement rates (Michelsen et al., 1986; Mariano et al., 2018), to inhibit dances and to reduce recruitment without discriminating for the foraging location (Kirchner, 1993). We go beyond these results by using vibration elements embedded into the comb to selectively suppress unwanted dances after their detection and decoding via technology. This unique combination of dance decoding, dance suppression, and a dance robot within the HIVEOPOLIS superorganism will reduce competition among the hives, preserve protected areas for other pollinators which are sometimes outcompeted by honeybees (Wojcik et al., 2018), and prevent bees from foraging at locations that are known to be detrimental for them, e.g., due to pesticides (Pettis et al., 2013; Meikle et al., 2016; Motta et al., 2018).
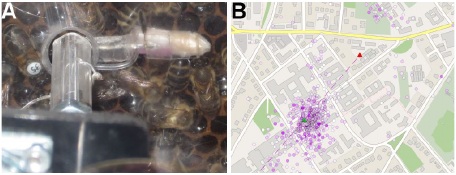
Across-Colony Models of Interaction.
An apiary of several beehives exchanging data forms a heterogeneous distributed bio-hybrid system. This bio-hybrid superorganism will be able to act at two distinct abstract levels. The global level incorporates an environmental information system that is responsible for collection, exchange of data (as shown in Fig. 1) and maintenance of the augmented map. At the local level bio-hybrid hives are able to communicate with each other in order to adjust the swarms’ behaviour.
Our intention is to stabilize and support the ecosystem by finding the ideal balance between cooperation and competition at the local level, utilizing data from the global level. Accordingly, we analyze the competition factors between colonies and develop an adaptive interaction model to identify configurations that lead to detrimental competition or cooperation. Specific optimisation goals could be pollination service or food distribution. The apiary level (several colonies) decision making and negotiation model will be crucial for allowing improved operational strategies at the level of the whole apiary, such as foraging from N spots by M colonies.
To study these dynamics, we introduce a simulation of the bio-hybrid superorganism within its ecosystem that implements several learning strategies such as reinforcement learning and predictive coding. In robotics, predictive coding is an important concept to facilitate anticipation and decision making in autonomous agents (Ciria et al., 2021; Mellmann et al., 2020). This novel approach considers the bio-hybrid hives as autonomous agents in a complex environment. The generated decision-making and negotiation models can then be used to analyze the adaptation ability of bio-hybrid hives and their populations to environmental factors and the current state of neighbouring hives as well as to define a set of tradeoffs between competing agents.
In future implementations, the information gained from the simulation can be directly used to adapt properties of the hives, or vice versa, thus even allowing bees to influence their hive’s location. This way, HIVEOPOLIS re-empowers colonies to have their say in their own housing decisions, like their wild ancestors tended to have (Seeley, 2010).
Augmented Environmental Maps.
Beekeepers are often confronted with crucial economic and ecological questions, such as: Where should they place their apiary? How many hives should be placed in one location? We have developed several models to evaluate the location of the apiary based on the theoretical availability of foraging resources and existing limitations (Komasilova et al., 2020). A model for real agricultural field location digitization and evaluation of possible apiary locations by fusing information about available field resources is proposed. To achieve this, several steps have to be completed, such as selecting fields of interest, converting them to polygons for further calculations, defining the potential values and coefficients for the amount of resources depending on type of crops and season and calculation of harvesting locations. Our model produces heat maps of possible apiary locations that are presented to the end-user visualized as an augmented environmental map. Based on this outcome, beekeepers can plan an optimal placement of the apiary or change it, if needed.
Another aspect of EH is the prediction and evaluation of the effect of meteorological factors on honeybee colony foraging behaviour. Foraging behaviour is one of the distinctive behaviours of honeybees and represents a link between the honeybee colony and the ambient environment. It is well known that environmental and meteorological factors can have either a positive or a negative impact on the foraging activity in general and the amount of gathered honey in particular. Within this part of the research, conclusions on the effect of different meteorological factors on colony foraging will be made and optimal foraging conditions will be identified. Besides its value to community stakeholders, this extracted information will inform our predictive models and control objectives used inside our augmented hives.
Opportunities and Limitations
Behavioural links: Social insects are especially suited as candidate organisms to augment their colony with technological artifacts. The social interactions within their selfregulation offer specific behavioural “hooks” to integrate technology, as these feedback loops involve environmental cues or direct communication. These mechanisms allow artificial agents (e.g., autonomous robots) to monitor the social context (input) or to influence (output) the insect society. This way the agents can participate in the colony’s self-regulating.
Technological links: After identifying suitable behavioural interfaces, the development of novel technologies that can perform observation and modulation of those behaviours is necessary, however strongly limited by the constraints and demands of the organism the technology has to interact with, in order to not create unwanted interferences or side effects.
Biocompatibility & Bioeffectivity: Biological acceptance of the technology enables natural behaviours to persist with minimal disturbance. With honeybees, material choices, nonintrusive sensing, and sources of heat or noise should all receive attention. The technology should also allow modifying information flows in the biological group, e.g., via biomimicry or modulation environmental factors. Key examples in HIVEOPOLIS include modulating foraging information through biomimetic mechatronics, and modulating the thermal environment including in the broodnest. The high-level control programs defining how and when to act are an essential element of a bio-hybrid system, and should be based on detailed knowledge and modelling (Mondada et al., 2013).
Conclusions and Open Challenges
Integrating technology into a meaningful and biocompatible way is a scientific challenge. We showed that it is a feasible path to monitor organisms for a proactive procurement and even to modulate their behaviours. In honeybees, this requires “rethinking” the very concept of a beehive and tackling the biohybrid- and social-integration on multiple layers.
The main message we take from the presented research is that the different layers of a honeybee colony’s distributed system of self-organization and the associated feedback-loops that process information, present many accessible “hooks” to insert technology into the information flow and thus, also regulatory control loops. We have demonstrated that our system can monitor environmental data (cues) in the brood nest area of the colony, such that we can observe brood growth and thus health development. We have implemented actuators for emitting temperature stimuli, vibration stimuli and airflow stimuli into the combs of the brood nest, for all of which it was shown that they are able to influence local densities of bees (Schmickl et al., in press; Szopek et al., in press; Bonnet et al., 2019; Mariano et al., 2018), and demonstrated the effect of added warmth onto the brood nest development.
To allow these technologies to find their place in the hive, we have to develop novel hive topologies that facilitate the ubiquitous interface between bees and technology in both ways. Our analysis of hive topologies has shown that both candidates, the “star” topology as well as the “mitochondria” topology, were well accepted by the bees. However, the “star” topology proved to be better performing in colony development and more suitable for implementing the technology needed to augment the colony, thus this topology will be our way to go forward. In order to do so, we developed a design recipe that employs A.I. methods to create naturally shaped housings for bees that are 3d-printable from organic materials. It uses waste materials as substrate for further growth via a fungus, akin to those tree fungi that occur around the bees’ natural living quarters, which are tree cavities. Other examined topologies, like the community hive, are useful for making HIVEOPOLIS technology accessible to the broader public, for research and for new bee breeding methods.
All design principles consider space for technology that allows environmental data and the predictions of forecast models to be incorporated. A specialized non-colony-invasive honey harvesting technology is also anchored in our design principles, in order to make the technology relevant for beekeepers of various scales, from private lifestyle-beekeepers to large-scale beekeepers and agriculture.
Environmental data collection, data sharing, and data influx into HIVEOPOLIS hives is targeted by augmented environmental maps, connecting the bee colony’s foraging behavior with the apiary surroundings and climate. These can inform the system to boost ecosystem service as well as to allow crucial protection of bees from dangerous or otherwise unwanted foraging sites. These functionalities are targeted by a novel combination of a biomimetic robot that imitates waggle-dances with an integrated dance analysis system, allowing both unique manipulations of a colony’s foraging efforts as well as communication between hives.
Thinking about the ethical perspective of our approach, either from an environmentalist or from an animal-welfare perspective, we identify two prominent and critical risks: Can such biohybrid systems start spreading in an uncontrolled manner? Can the bees become too dependent on our technology? The first risk is averted by our choice of honeybees as the target animals. Honeybees spread by swarming, which is colony division. Even if the bees leave the hive in a reproductive swarm, the technological augmentation of the hive will stay in place. Other alternative approaches to counteract the trend towards natural diversity loss, like genetically altered or imported species, pose a much higher risk to spread and get out of control. The second risk is more plausible to happen, however, honeybees are basically agricultural livestock. Thus, the increase of dependency on human care over domestication time is always a given factor.
In the future, we will benchmark the integrated system that we have so far only tested as modular subparts. We demonstrate and showcase here first prototypes, with the first evaluations and benchmarks concerning their effects on the colony performance metrics, like productivity and robustness. Many novel technologies, from electronics to materials, from software to mathematical models, will be needed to be further developed in the upcoming years, in order to establish this HIVEOPOLIS system as an autonomously running biohybrid, as an autonomously operating biophysical implementation of ALIFE on the super-organismic level.
References
Belsky, J., & Joshi, N. K. (2019). Impact of biotic and abiotic stressors on
managed and feral bees. Insects, 10(8): 233.
Bonnet. (2017). Design of a modular robotic system that mimics small fish
locomotion and body movements for ethological studies. Int. J. Adv. Robot. Syst. https://doi.org/10.1177/1729881417706628
Bonnet, F., Mills, R., Szopek, M., Schönwetter-Fuchs, S., Halloy, J.,
Bogdan, S., Correia, L., Mondada, F., and Schmickl, T. (2019). Robots mediating interactions between animals for interspecies collective behaviors. Science Robotics, 4(28):eaau7897.
Butler, Z., Corke, P., Peterson, R., & Rus, D. (2006). From Robots to
Animals: Virtual Fences for Controlling Cattle. The International Journal of Robotics Research, 25(5–6), 485–508.
https://doi.org/10.1177/0278364906065375
Cavalier-Smith, T. (2006). Cell evolution and Earth history: stasis and
revolution. Philosophical Transactions of the Royal Society B: Biological Sciences, 361(1470): 969-1006.
Ciria, A., Schillaci, G., Pezzulo, G., Hafner, V.V., and Lara, B. (2021).
Predictive Processing in Cognitive Robotics: a Review. Neural Computation 2021. 33 (5): 1402–1432.
Elsacker, E., Poncelet, W., Peeters, E., and De Laet, L. (2019).
Transdisciplinary Knowledge Sharing Platform for Biologically Augmented Materials for the Building Industry. In IASS Annual Symposium 2019 – Structural Membranes 2019 Form and Force, Barcelona, Spain.
EC (European Commission) (2019). Futuristic Beehives for a Smart
Metropolis. https://cordis.europa.eu/project/id/824069
Faria, J. J., Dyer, J. R. G., Clément, R. O., Couzin, I. D., Holt, N., Ward,
A. J. W., Waters, D., & Krause, J. (2010). A novel method for investigating the collective behaviour of fish: Introducing ‘Robofish’. Behavioral Ecology and Sociobiology, 64(8), 1211–1218.
https://doi.org/10.1007/s00265-010-0988-y
Fukuda, H. (1971). Improvement of Bodenheimer’s Method for Estimating
Individual Number in Honeybee Colonies. Jour. Fac. Sci. Hokkaido Univ. Ser. VI, Zoot, 18(1): 128-143.
Gould, S. J. (1994). The evolution of life on the earth. Scientific American,
271(4): 84-91.
Griparic, K., Haus, T., Miklic, D., & Bogdan, S. (2015). Combined actuator
sensor unit for interaction with honeybees. 2015 IEEE Sensors Applications Symposium (SAS), 1–5.
https://doi.org/10.1109/SAS.2015.7133604
Halloy, J., Sempo, G., Caprari, G., Rivault, C., Asadpour, M., Tache, F.,
Said, I., Durier, V., Canonge, S., Ame, J. M., Detrain, C., Correll, N.,
Martinoli, A., Mondada, F., Siegwart, R., & Deneubourg, J. L. (2007).
Social Integration of Robots into Groups of Cockroaches to Control Self-Organized Choices. Science, 318(5853), 1155–1158.
https://doi.org/10.1126/science.1144259
Harding, J., and Brandt-Olsen, C. (2018) Biomorpher: Interactive Evolution for Parametric Design. International Journal of Architectural Computing 16(2): 144–63.
Hobbs, C. (2005). Medicinal Value of Turkey Tail Fungus Trametes versicolor (L.:Fr.) Pilat (Aphyllophoromycetideae). International Journal of Medicinal Mushrooms, 7(3): 346–347.
Ilgun, A. and Ayres, P. (2016). Self-Organised Embellishment of 3D Printed Scaffolds for the Production of Co-occupied Architectural Boundaries. In 2016 IEEE 1st International Workshops on Foundations and Applications of Self* Systems (FAS*W), Augsburg, Germany, pages 143-144.
Jong, E. D., Field, J. A. (1997). SULFUR TUFT AND TURKEY TAIL: Biosynthesis and Biodegradation of Organohalogens by Basidiomycetes. Annual Review of Microbiology, 51(1): 375–414.
Kirchner, W. H. (1993). Vibrational signals in the tremble dance of the honeybee, Apis mellifera. Behavioral Ecology and Sociobiology, 33(3): 169–172.
Komasilova, O., Komasilovs, V., Kviesis, A., Bumanis, N., Mellmann, H., and Zacepins, A. (2020). Model for the bee apiary location evaluation. Agronomy Research, 18(S2): 1350-1358.
Landgraf, T. (2013). RoboBee: A Biomimetic Honeybee Robot for the Analysis of the Dance Communication System [Berlin, Freie Universität Berlin, 2013].
http://www.diss.fuberlin.de/diss/receive/FUDISS_thesis_000000094818?lang=de
Landgraf, T., Bierbach, D., Kirbach, A., Cusing, R., Oertel, M., Lehmann, K., Greggers, U., Menzel, R., and Rojas, R. (2018). Dancing Honey bee Robot Elicits Dance-Following and Recruits Foragers.
ArXiv:1803.07126 [Cs]. http://arxiv.org/abs/1803.07126
Langton, C. G. (1990). Computation at the edge of chaos: Phase transitions and emergent computation. Physica D: Nonlinear Phenomena, 42(1-3): 12-37.
Levin, S. A. (1998). Ecosystems and the biosphere as complex adaptive systems. Ecosystems, 1(5): 431-436.
Lewin, R. (1999). Complexity: Life at the edge of chaos. University of Chicago Press
Lombard, J. (2014). Once upon a time the cell membranes: 175 years of cell boundary research. Biology direct, 9(1): 1-35.
Maynard Smith J., Szathmary E. (1995). The Major Transitions in Evolution. San Francisco: W. H. Freeman
Mariano, P., Salem, Z., Mills, R., Schönwetter-Fuchs-Schistek, S., Correia, L., & Schmickl, T. (2018). Evolving robot controllers for a bio-hybrid system. The 2018 Conference on Artificial Life.
https://doi.org/10.1162/isal_a_00036
McCoy E.D., Bell S.S. (1991) Habitat structure: The evolution and diversification of a complex topic. In: Bell S.S., McCoy E.D., Mushinsky H.R. (eds) Habitat Structure. Population and Community Biology Series, vol 8. Springer, Dordrecht.
Meikle, W. G., Adamczyk, J. J., Weiss, M., Gregorc, A., Johnson, D. R., Stewart, S. D., Zawislak, J., Carroll, M. J., and Lorenz, G. M. (2016). Sublethal Effects of Imidacloprid on Honey Bee Colony Growth and Activity at Three Sites in the U.S. PLOS ONE, 11(12):e0168603.
Meikle, W., & Holst, N. (2015). Application of continuous monitoring of honeybee colonies. Apidologie, 46(1), 10–22.
https://doi.org/10.1007/s13592-014-0298-x
Mellmann, H., Schlotter, B., Musiolek, L., and Hafner, V.V. (2020).
Anticipation as a Mechanism for Complex Behavior in Artificial Agents.
In ALIFE2020, The 2020 Conference on Artificial Life, pages 157-159. MIT
Press.
Michelsen, A., Kirchner, W. H., Andersen, B. B., and Lindauer, M.
(1986). The tooting and quacking vibration signals of honeybee queens: A
quantitative analysis. Journal of Comparative Physiology, 158:605–611.
Michelsen, A., Andersen, B. B., Storm, J., Kirchner, W. H., &
Lindauer, M. (1992). How honeybees perceive communication dances,
studied by means of a mechanical model. Behavioral Ecology and
Sociobiology, 30(3–4), 143–150. https://doi.org/10.1007/BF00166696
Mondada, F., Martinoli, A., Correll, N., Gribovskiy, A., Halloy, J.
I., Siegwart, R., and Deneubourg, J.-L. (2013). A general methodology
for the control of mixed natural-artificial societies. In Handbook of
Collective Robotics, Chapter 15, pp. 547–586). Pan Stanford Publishing.
Motta, E. V. S., Raymann, K., and Moran, N. A. (2018). Glyphosate perturbs the gut microbiota of honey bees. Proceedings of the National Academy of Sciences, 115(41):10305–10310.
Oxman, N. (2007). Digital Craft Fabrication-Based Design in the Age of Digital Production. In: Procs International Conference on Ubiquitous Computing (Ubicomp 2007), pages 534-538
Parmee, I. C. (1996). Cluster oriented genetic algorithms (cogas) for the identification of high performance regions of design spaces. In First International Conference on Evolutionary Computation and Applications, EvCA, 96:66–75.
Pettis, J. S., Lichtenberg, E. M., Andree, M., Stitzinger, J., Rose, R., and van Engelsdorp, D. (2013). Crop Pollination Exposes Honey Bees to Pesticides Which Alters Their Susceptibility to the Gut Pathogen Nosema ceranae. PLOS ONE, 8(7):e70182.
Potts, S. G., Imperatriz-Fonseca, V., Ngo, H. T., Aizen, M. A., Biesmeijer, J. C., Breeze, T. D., Dicks, L. V., Garibaldi, L. A., Hill, R., Settele, J., and Vanbergen, A. J. (2016). Safeguarding pollinators and their values to human well-being. Nature, 540(7632):220-229.
Ribatti, D. (2018). An historical note on the cell theory. Experimental cell research, 364(1):1-4.
Romano, D., Donati, E., Benelli, G., & Stefanini, C. (2019). A review on animal–robot interaction: From bio-hybrid organisms to mixed societies. Biological Cybernetics, 113(3), 201–225.
https://doi.org/10.1007/s00422-018-0787-5
Schmickl, T., Szopek, M., Mondada, F., Mills, R., Stefanec, M., Hofstadler D. N., Lazic, D., Barmak, R., Bonnet, F., and Zahadat, P. (in press). Social integrating robots suggest mitigation strategies for ecosystem decay. To appear in Frontiers in Bioengineering and Biotechnology.
Seeley, T. D. (2010). Honeybee democracy. Princeton University Press.
Stabentheiner, A., Kovac, H., and Brodschneider, R. (2010). Honeybee colony thermoregulation–regulatory mechanisms and contribution of individuals in dependence on age, location and thermal stress. PLoS One, 5(1):e8967.
Stamets, P. (2005). Mycelium Running: How Mushrooms Can Help Saving the World? Random House Digital, Inc. Stefanec, M., Szopek, M., Schmickl, T., & Mills, R. (2017). Governing the swarm: Controlling a bio-hybrid society of bees robots with computational feedback loops. 2017 IEEE Symposium Series on Computational Intelligence (SSCI), 1–8.
https://doi.org/10.1109/SSCI.2017.8285346
Szopek, M., Thenius, R., Stefanec, M., Hofstadler, D., Varughese, J., Vogrin, M., Radspieler, G., and Schmickl, T. (in press). Autonome Roboterschwärme als Stabilisatoren gefährdeter Ökosysteme. To appear in Navigationen – Multispecies Communities.
Timmis, J., Hone, A., Stibor, T., and Clark, E. (2008). Theoretical advances in artificial immune systems. Theoretical Computer Science, 403(1):11-32.
Turner, T. (2017, July 4). A buzzworthy beehive. Retrieved from
https://www.yankodesign.com/2017/04/07/a-buzzworthy-beehive/
Vaughan, R., Sumpter, N., Henderson, J., Frost, A., & Cameron, S. (2000). Experiments in automatic flock control. Robotics and Autonomous Systems, 31(1–2), 109–117. https://doi.org/10.1016/S0921-8890(99)00084-6
von Frisch, K. (1965). Tanzsprache und Orientierung der Bienen. Springer.
http://www.springer.com/us/book/9783642949173
Wario, F., Wild, B., Rojas, R., and Landgraf, T. (2017). Automatic detection and decoding of honey bee waggle dances. PLOS ONE, 12(12):e0188626.
Wojcik, V. A., Morandin, L. A., Davies Adams, L., and Rourke, K. E. (2018). Floral Resource Competition Between Honey Bees and Wild Bees: Is There Clear Evidence and Can We Guide Management and Conservation? Environmental Entomology, 47(4):822–833.
Worm, M., Kirschbaum, F., & Emde, G. von der. (2017). Social interactions between live and artificial weakly electric fish: Electrocommunication and locomotor behavior of Mormyrus rume proboscirostris towards a mobile dummy fish. PLOS ONE, 12(9), e0184622.
https://doi.org/10.1371/journal.pone.0184622
Yang, Z, Zhang, F., Still, B., White, M., and Amstislavski, P. (2017) Physical and Mechanical Properties of fungal Mycelium-Based Biofoam. Journal of Materials in Civil Engineering, 29:04017030.
Youngsteadt, E., Appler, R. H., López-Uribe, M. M., Tarpy, D. R., and Frank, S. D. (2015). Urbanization increases pathogen pressure on feral and managed honey bees. PloS one, 10(11): e0142031.